Engineered cell therapies an emerging branch of medicine
Biology is being rapidly transformed from an analytical to a synthetic, engineering discipline. For millennia, we have observed, described, and studied animals, plants, and microbes. We have tinkered with them for sure, and in many instances with remarkable success.
For example, by breeding and selecting for the desired traits in agricultural crops and animals, we have secured the food supply for several billion people; however, tinkering is slow and relatively inefficient. The progress in molecular, cellular, and developmental biology over the past few decades has enabled much more precise, fast, and efficient manipulation of genetic programs.
We now have the ingredients for reprogramming cells and organisms with increasing predictability of the outcomes. We are at the dawn of a bioengineering revolution. While no one can envision its full scope, engineered cell-based therapies give us a glimpse of what is coming.
The year 2017 was remarkable because the year marked the first approval of an engineered cell therapy. This involves isolating a patient’s T cells (a type of white blood cell part of the immune system) and reprogramming them in the laboratory so they can recognize and destroy specific tumor cells. The engineered T cells hunt and kill tumor cells upon injection in the bloodstream. Thousands of people with leukemia and lymphoma have been saved or their lives extended by the T cells that have been transformed into better tumor assassins.
Killing is not the only trick we can teach human cells. In regenerative medicine, cells are programmed to become better healers of damaged organs. Yet another emerging therapy relies on engineering cells to produce genetically encoded drugs. Such cells may be implanted under the skin or elsewhere in the body and work as miniature pharmaceutical factories patients carry with them.
Imagine a person with diabetes whose implanted cells produce insulin, or a severely obese individual whose implanted cells produce appetite-suppressing hormones and help in decreasing food portions, or a patient with a neurological disorder whose implanted cells produce a therapeutic neuropeptide.
How can we control engineered cells inside the human body?
We need to control activities of the implanted cells to advance engineered cell therapies, but how do you send commands to cells? Students in my laboratory have been developing light-based cell communication devices. Such devices work similar to TV remote controllers: a beam of near-infrared light reaches a TV set where embedded photodiodes turn an electric circuit on or off.
We like light as a means of controlling cell activity because light has no side effects; light is easy to turn on and off and can be focused on a specific body location.
Near-infrared light is perfect; it penetrates human tissues much deeper than visible light (Figure 1). If we program cells to interpret near-infrared light signals, we can control their activity inside the body using external lasers or LEDs. Since human cells don’t have near-infrared light sensors, we need to engineer and incorporate them into the existing cellular circuitry. Alternatively, we could engineer both light-sensing devices and a new circuitry through which to control cell activity.
We have made significant progress in both pursuits, but the road to success has been long and arduous.
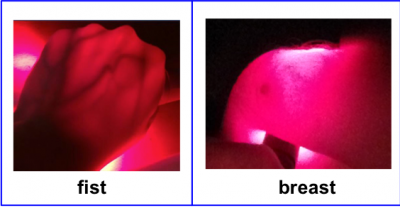
Humans don’t have photoreceptors for the near-infrared spectrum, but some bacteria do. They use photoreceptor proteins known as bacteriophytochromes to detect light in the 700-770 nanometer region, at the border of red and near-infrared (Figure 2). While studying how the photosynthetic bacterium Rhodobacter responds to light, postdoctoral fellow Marina Tarutina, and Ph.D. student Dmitry Ryjenkov, both from Russia, have identified and characterized its bacteriophytochrome proteins. This was almost 15 years ago. Back then we projected that one day bacteriophytochromes would be engineered to control mammalian cells (see inset below).
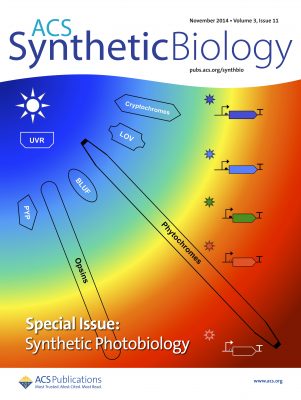
How to program cells to see near-infrared light?
The diligence and perseverance of another Ph.D. student, Min-Hyung Ryu from South Korea, brought that vision to fruition. Min-Hyung has undertaken a daring protein engineering project to construct a bacteriophytochrome with an artificial, adenylate cyclase, activity.
The bacteriophytochrome engineering method we have invented is now protected by patents owned by UW. Because an adenylate cyclase naturally controls various activities in animal cells, an engineered protein could be plugged into the existing cell circuitry, which we can now control using light. Our collaborators from the University of Pennsylvania Medical School put an engineered protein into the neurons of a worm, C. elegans, to control swimming behavior of this tiny animal using light. Other collaborators tried to control the behavior of mice, the next animal model in developing biomedical applications. Unfortunately, the engineered protein did not work in mice.
Disappointed yet defiant, we went back to the drawing board to methodically analyze what went wrong. Many things could potentially go wrong because bioengineering is not yet as straightforward as, for example, electrical engineering.
First, genetic parts are not nearly as standard as transistors or electric switches.
Second, our understanding of cellular circuitry is not nearly as complete as that of electrical circuitry.
Last but not least, cellular circuitry is much more complex than any electric one.
Still, we determined the cause of our initial failure.
The genetic parts we used to engineer the original protein were taken from bacteria that cannot grow at human or mouse body temperature. Not surprisingly, the engineered protein worked well in worms at ambient temperature but failed to work in warm-blooded mice.
Another talented Ph.D. student, Anastasia Fomicheva from Russia, remade the photoactivated adenylate cyclase using genetic parts from ‘tougher’ bacteria. She showed that the next-generation protein can be produced by human cells grown in culture and works well at 100° F.
The students of Professor Qian-Quan Sun, our collaborator from the Department of Zoology and Physiology and director of the Sensory Biology Center, have now used this next-generation protein to control neuronal activity in mice using light. Brain neurons producing our protein can be turned on by using a hand-held, near-infrared laser. Importantly, laser light penetrates through the mouse skull and brain tissue causing no damage.
Before leaving for a postdoctoral fellowship at MIT, Min-Hyung designed yet another bacteriophytochome-based system with its own genetic circuitry. He perfected this system in E. coli cells, but this system can be used to control diverse cell types.
Chinese and Swiss bioengineers led by Professor Ye adapted our system for controlling production of insulin in human cells in response to light. Diabetic mice with implanted engineered cells were exposed to near-infrared light daily. During irradiation, the photoactivated cells produced insulin. The researchers calculated duration of irradiation daily based on blood glucose measurements. These diabetic mice have had blood glucose levels within the normal range for several weeks. That study was a remarkable demonstration of the power of photoactivated cell therapies.
Since then, two masters students in my laboratory, Marina Lazic from Serbia and Taylor Doherty from Laramie, have improved on Min-Hyung’s system. Ph.D. student Tasneem Muna from Bangladesh, in collaboration with the laboratory of Professor Jared Bushman from the School of Pharmacy, is adapting that system to control proliferation of T regulatory cells. Our goal is to develop photoactivated T regulatory cells that will maintain light-controlled immunosuppression.
We expect it to help prevent rejection of transplanted organs in survivors of severe injuries and burns, common among war veterans.
A path to photoactivated cell therapies
Will future patients irradiate their implanted cells while watching their favorite Netflix shows or during sleep?
Will LED wristbands that irradiate pouches of implanted cells become a new fashionable trend?
I can’t say.
Predicting fashion trends and social behavior is an exercise in futility. I do hope our bioengineering efforts will help progression of photoactivated cells toward therapeutic applications and that it happens in our lifetime.
Author: Mark Gomelsky, Professor, Department of Molecular Biology
To contact: Gomelsky can be reached at (307) 766-3522 or gomelsky@uwyo.edu.
What has made a bioengineering revolution possible?
Bioengineering has become possible as a result of several lines of progress. One of them is genomics, the field of science involved in determining genetic codes of different organisms. Not only has genomics brought us deeper understanding of genome structure, it has also exposed the breadth of genetic diversity in nature, that is the inventory of genetic parts available for engineering. Significant progress has been achieved in engineering of molecular tools with which we can precisely manipulate the genetic code – change existent genes, remove them, or add new genes. DNA synthesis technology has enabled fast and cheap gene synthesis based on the written code. The progress in biochemistry has illuminated functions of numerous proteins, the main molecular machines of a cell. Cell biology has explained how these proteins work together. The progress in developmental biology has taught how cells form organs, so we can reproduce natural genetic programs and design new ones. We understand reasonably well the genetic parts that code the components of the cellular machinery and have access to a myriad of genetic parts. We know the rules regulating how they interact and how cell fate is determined, plus we have the tools to modify genetic parts and to synthesize new ones. These are the prerequisites for successful bioengineering. We are at the dawn of the bioengineering revolution that will transform the bioworld around us and most likely us humans too.
From a bacteriologist studying light sensing to a bioengineer developing photoactivated cell therapies.
Science moves in unpredictable ways, simply because discoveries cannot be planned. When I started my laboratory at UW, we were interested in understanding how a photosynthetic bacterium Rhodobacter sphaeroides adapts to changes in oxygen and light. Our search for sensors for these environmental factors has led us to the discovery (with other scientists) of a new type of blue light sensor (which we named BLUF). We found that, in addition to a blue light sensor, Rhodobacter has a gene for a sensor of red or near-infrared light whose function was unknown. That seemingly obscure photoreceptor, bacteriophytochrome, inspired our thinking about regulating human cells with light. Since the Rhodobacter bacteriophytochrome could not regulate mammalian cells in its native form, we had to modify it. Once our protein engineering project had succeeded (after years of failure), we wanted to test the engineered protein in human cells, although we had not worked with such cells before. One thing led to another, ultimately transforming my basic science lab interested in environmental sensing in bacteria into a bioengineering/ synthetic biology lab that uses bacterial genetic parts for solving biomedical problems. I am sure our expertise in light sensing and bacteriology makes us better engineers of photoactivated cells.
Do you want to help develop photoactivated cell therapies?
Eos LABS (www.eosbiosystems.com) is a recently formed startup company whose goal is to develop and promote engineered photoactivated cell therapies. LABS stands for light-activated biosystems, and Eos is the name of the Greek goddess of the dawn. If you are excited about photoactivated cells and would like to contribute your molecular expertise, entrepreneurial skills, or financial resources to moving photoactivated cells toward therapeutics, we would like to hear from you.